Translate this page into:
From microscopes to molecules: The evolution of prostate cancer diagnostics
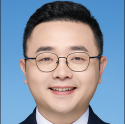
*Corresponding authors: Meng Zhang, Department of Urology, The First Affiliated Hospital of Anhui Medical University, Hefei, Anhui, China. zhangmeng1930@126.com
Jun Zhou, Department of Urology, The First, Affiliated Hospital of Anhui Medical University, Hefei, Anhui, China. urodoctorzhou@163.com
-
Received: ,
Accepted: ,
How to cite this article: Tao J, Bian X, Zhou J, Zhang M. From microscopes to molecules: The evolution of prostate cancer diagnostics. CytoJournal. 2024;21:29. doi: 10.25259/Cytojournal_36_2024
Abstract
In the ever-evolving landscape of oncology, the battle against prostate cancer (PCa) stands at a transformative juncture, propelled by the integration of molecular diagnostics into traditional cytopathological frameworks. This synthesis not only heralds a new epoch of precision medicine but also significantly enhances our understanding of the disease’s genetic intricacies. Our comprehensive review navigates through the latest advancements in molecular biomarkers and their detection technologies, illuminating the potential these innovations hold for the clinical realm. With PCa persisting as one of the most common malignancies among men globally, the quest for early and precise diagnostic methods has never been more critical. The spotlight in this endeavor shines on the molecular diagnostics that reveal the genetic underpinnings of PCa, offering insights into its onset, progression, and resistance to conventional therapies. Among the genetic aberrations, the TMPRSS2-ERG fusion and mutations in genes such as phosphatase and tensin homolog (PTEN) and myelocytomatosis viral oncogene homolog (MYC) are identified as significant players in the disease’s pathology, providing not only diagnostic markers but also potential therapeutic targets. This review underscores a multimodal diagnostic approach, merging molecular diagnostics with cytopathology, as a cornerstone in managing PCa effectively. This strategy promises a future where treatment is not only tailored to the individual’s genetic makeup but also anticipates the disease’s trajectory, offering hope for improved prognosis and quality of life for patients.
Keywords
Prostate cancer
Molecular diagnosis
Biomarkers
Liquid biopsy
Generation sequencing
INTRODUCTION
Prostate cancer (PCa) is the second most common malignancy among men globally, second only to lung cancer.[1] Forecasts for 2023 predict approximately 288,300 new PCa cases in the United States, accounting for 29% of all male cancer diagnoses and resulting in approximately 34,700 deaths, or 11% of all male cancer-related deaths,[2] posing a significant health and economic burden on men. Clinical staging through the tumor-node-metastasis system and pathological grading using the Gleason score have long served as the foundation for assessing PCa prognosis and guiding treatment.[3,4] Treatment options for localized PCa include active surveillance, radical surgery, or radiation therapy; however, up to 30% of patients still experience recurrence and metastasis following these treatments.[5] For advanced and metastatic PCa, androgen deprivation therapy (ADT), aimed at inhibiting androgen receptor (AR) signaling, is the primary strategy. Nevertheless, most patients who undergo ADT progress to a castration-resistant phase, termed castration-resistant PCa (CRPC), for which effective treatments are currently lacking.[6,7] Thus, early and precise assessment and management of the tumor are imperative.
A major challenge in the clinical management of PCa is insufficient early characterization of the tumor. Current diagnostic practices primarily rely on cytopathological examination, namely, microscopic analysis of prostate tissue biopsy samples, to characterize tumors. This allows for the direct identification of cancer cells and their morphological traits, whereas the Gleason grading system assesses tumor differentiation,[8] offering vital insights into disease status and prognosis. However, with the advancement of tumor molecular research, the critical role of the genetic heterogeneity of PCa in its development and progression has become increasingly acknowledged. The genetic heterogeneity of PCa signifies that even in the early stages, morphological patterns can vary significantly among different patients. Within a single tumor, cells displaying similar morphological characteristics can possess extensive variations in gene expression and mutations, influencing the tumor’s growth rate, aggressiveness, and treatment response.[9]
While cytopathological examination provides essential background information on cell morphology, it relies on molecular diagnostic methods to capture genetic heterogeneity and more precisely characterize cancer. In the future, an important trend in PCa management will be the integration of cytopathology with molecular diagnostics through a multimodal diagnostic approach. Cytology continues to play a crucial role in determining the morphological features of tumors and provides suitable cellular material for genetic and molecular analysis, while molecular diagnostics offer in-depth molecular details, such as specific gene mutations,[9] gene fusions,[10] epigenetic changes,[11] and non-coding RNAs.[12] By detecting these variations, they uncover the molecular mechanisms of cancer and identify potential therapeutic targets, offering crucial information for early diagnosis, prognosis assessment, and treatment decision-making in PCa.
This review summarizes the latest research advancements in molecular diagnostics for PCa, focusing on cutting-edge molecular biomarkers and associated detection technologies, and reaffirms the indispensable value of cytology in supporting the interpretation of molecular diagnostic findings and obtaining high-quality samples for molecular diagnostics.
GENETIC BIOMARKERS
TMPRSS2-ERG fusion
TMPRSS2, a gene responsive to androgens, produces a serine protease unique to the prostate, exhibiting specificity to prostate tissue.[13] Its operational significance hinges on the gene’s fusion with E-twenty six (ETS) transcription factors, such as ETS-Related Gene (ERG), which is a transcription factor with oncogenic potential.[14-16] The TMPRSS2:ERG gene fusion represents the most common genetic modification in PCa, occurring in about half of all PCa cases.[14-16] This particular fusion leads to the increased expression of ERG, contributing to the observed upsurge in cell proliferation, angiogenesis, and invasion of PCa cells.[17]
In recent years, the TMPRSS2:ERG fusion has been identified as an emerging biomarker for PCa, offering new avenues for diagnosis and understanding the disease’s pathology.[10,18] According to Mosquera et al.,[19] TMPRSS2-ERG fusion transcripts occur within prostatic intraepithelial neoplasia, and their expression levels correlate with the malignancy of PCa, improving the positive predictive value of finding TMPRSS2-ERG fusion PCa in subsequent biopsies, marking a significant advance in identifying precancerous lesions of PCa.
At the same time, many studies have emphasized the value of detecting TMPRSS2-ERG fusion in urine samples. Molecular genetic analysis of exfoliated prostate epithelial cells in urine samples to detect the levels of TMPRSS2:ERG fusion transcripts, it may offer advantages over serum prostate specific antigen (PSA) in some aspects of PCa diagnosis.[20] For example, a test developed by Mlabs of the University of Michigan for urine samples post-digital rectal examination (DRE), termed the Mi-Prostate Score, revealed that the sensitivity of TMPRSS2-ERG ranges from 24.3% to 37%, and its specificity reaches 93%.[21] Traditional PSA tests have a specificity ranging from 20% to 40%, thus, the heightened specificity of the TMPRSS2:ERG fusion acts as an effective adjunct to PSA. Notably, Rice et al.[22] analyzed ERG mRNA in the urine of 237 men and demonstrated the 80% predictive accuracy of the TMPRSS2:ERG fusion in diagnosing PCa in men with PSA levels ≤4.0 ng/mL.
Recent research has highlighted the combined diagnostic significance of TMPRSS2:ERG and prostate cancer antigen 3 (PCA3) in urine samples. The findings indicate that integrating urinary PCA3 and TMPRSS2-ERG levels with serum PSA substantially improve the detection of aggressive PCa (characterized by a Gleason score ≥ 7) at the time of the initial biopsy. Moreover, this combined diagnostic approach has been shown to decrease the number of unnecessary biopsies by 42%.[23-25]
PTEN loss
The PTEN gene, known for its tumor-suppressing functions, facilitates cell apoptosis by inhibiting activation of the phosphoinositide 3-kinase/protein kinase B(PI3K/AKT) signaling pathway, which is crucial for maintaining regular cell growth and preventing tumor development.[26,27] Studies have shown that alterations in the PTEN gene, including copy number variations, structural rearrangements, and point mutations, lead to its deactivation. These changes are present in approximately 20% of primary PCa specimens and in 50% of CRPC cases, culminating in a loss of its tumor-suppressive abilities.[28,29]
Extensive research has demonstrated a strong correlation between the frequency of PTEN inactivation and more aggressive PCa features, worse prognosis, and diminished responsiveness to conventional endocrine therapy.[30-32] Significantly, liquid biopsy analysis based on the PTEN genomic status has been applied clinically as a rapid, noninvasive strategy to provide information for treatment decisions. In a clinical trial exploring the combination of abiraterone with PI3K/AKT inhibitors in CRPC patients, researchers used blood-based circulating tumor cells (CTCs) PTEN fluorescence in situ hybridization testing and found that PTEN-deficient CRPC patients had significantly reduced survival rates and markedly affected responses to current therapies.[33,34]
Moreover, in localized PCa, the status of the PTEN gene has been recognized as a critical predictive marker for the progression of the disease, aiding in the selection of intervention timing for patients under active surveillance. For example, through immunohistochemical examination of PTEN in biopsy samples, researchers found that PTEN loss in localized PCa is associated with an increased risk of non-organ-confined PCa, indicating that these patients may benefit more from radiation therapy or radical prostatectomy.[35,36]
MYC amplification
The MYC gene is intimately linked with numerous cancers and tumor-specific traits. In studies across 33 cancer types encompassing genomics and proteomics, MYC gene amplification was identified in 28% of tumor samples, underscoring its widespread impact.[37] As a distinct oncogenic driver, the MYC gene encodes a nuclear DNA-binding protein pivotal for cell cycle regulation. This gene and its outputs foster cell proliferation, immortalization, dedifferentiation, and transformation, thereby accelerating tumor initiation and progression.[38,39] These features highlight the potential of MYC as both a therapeutic target and a biomarker.
Reports indicate that amplification of the Myc gene is observed in 89% of PCa patients.[40] Amplification of the MYC gene is linked to increased Gleason scores, increased disease aggressiveness, and reduced survival in PCa patients, serving as a key driver of disease worsening and metastasis.[41-43] Research by Zhang et al.[44] has shown that overexpression of MYC disrupts the transcriptional program of the AR, which plays a pivotal role in PCa development. This indicates that elevated levels of MYC amplification could accelerate the progression of PCa to metastatic and castration-resistant phases. Local radiotherapy stands as an essential treatment for the early-stage PCa. Investigations have highlighted a link between variations in c-Myc copy number in PCa and genomic instability. Notably, PCa patients exhibiting high c-Myc expression who receive radiotherapy are at increased risk of disease recurrence and metastasis compared to those with lower c-Myc expression, indicating that radiotherapy might be less effective in these individuals.[45] Utilizing cytological methods such as liquid biopsy to monitor MYC amplification could aid in identifying patients who might benefit from alternative treatments or combination therapies, thereby improving personalized treatment plans and outcomes.
AR mutation and amplification
During the development of PCa to CRPC, the primary driver is aberrant activation of the AR signaling pathway. This involves mechanisms such as AR mutations,[46] amplification of the AR gene and its enhancers,[47] and the emergence of AR splice variants (AR-Vs),[48] rendering PCa sensitive to low androgen levels.[49]
Reports reveal that approximately 80% of CRPC patients exhibit AR overexpression, and 30–50% of their tissue samples exhibit AR gene amplification.[50] Moreover, nearly 60% of metastatic PCa patients have CTCs showing AR gene amplification and mutation.[51] Although the prognostic implications of AR amplification and mutations are not fully understood, they are recognized as negative prognostic indicators in the circulating cell-free DNA (cf-DNA) of metastatic CRPC (mCRPC) patients.[52-54]
At present, current attention is focused on AR gene structural rearrangements or alternative splicing that produce ARVs, such as the classic AR-V7.[55] A recent systematic review and meta-analysis conducted by Khan et al.[56] evaluated the clinical relevance of AR-V7 detection in liquid biopsy (primarily blood), showing that the presence of AR-V7 in such biopsies is an important clinical biomarker associated with overall survival (OS), progression-free survival (PFS), and PSA-PFS in CRPC patients receiving AR antagonist therapy. Detecting AR-V7 in liquid biopsy from CRPC patients has prognostic and predictive capabilities, a finding with high clinical relevance. At present, tests for analyzing AR-V7 status in blood CTCs have been commercialized and can be used in clinical practice, facilitating the assessment of AR-V7 status in patients with CRPC and highlighting the value of fluid biopsy in providing crucial prognostic and treatment-related information.[57]
EPIGENETIC BIOMARKERS
DNA methylation
DNA methylation, the addition of methyl groups to DNA molecules, can result in the silencing of genes and decreased expression within promoter regions. This prevalent type of epigenetic alteration is linked to the development of multiple cancer types.[58-61] Considering the high incidence of abnormal DNA methylation in PCa-surpassing the frequency of genetic alterations-DNA methylation patterns have emerged as promising candidate biomarkers.[62,63]
In their research, Kim et al.[64] examined tissue samples from 53 patients who had undergone radical prostatectomy, comprising 42 cases of PCa and 11 cases of prostatic hyperplasia. Their findings demonstrated that the prognostic statistical significance of increased and decreased methylation area under the curve (AUC) values were 0.99 and 0.98, respectively, which were markedly higher than the 0.79 associated with PSA. This finding suggested that DNA methylation assessment could offer a more effective diagnostic method for PCa compared to the conventional PSA test.
The methylation of glutathione S-transferase P1 (GSTP1) is common in PCa, impacting about 90% of patients. It functions as an epigenetic biomarker for the early cancer detection.[65] The methylation-specific polymerase chain reaction (MSP) method can successfully detect GSTP1 methylation in CTCs and cf-DNA in blood samples. In addition, highly specific quantitative MSP methods can detect GSTP1 methylation in tissue biopsy samples to accurately differentiate between benign and malignant prostate lesions.[66] Based on this method, commercially available tests have been developed and are primarily used for clinical assessment of the necessity for repeat prostate biopsies.[67,68]
Identifying DNA methylation biomarkers through measurement aids in risk stratification among PCa patients. For example, the methylation status of specific genes, such as high methylation of actin-like 6B and low methylation of transcription elongation regulator 1, is associated with aggressive tumor traits and poor prognosis. These methylation patterns can serve as independent predictors of recurrence in localized PCa,[69] potentially guiding more aggressive treatment for individuals identified as high-risk due to these methylation patterns. Methylation analysis further serves as a diagnostic measure for uncommon variants of PCa. Berchuck et al.[70] constructed a predictive framework predicated on variations in methylation across different types of PCa. This model was utilized on a group of 48 patients, consisting of nine cases of neuroendocrine PCa and 39 cases of castration-resistant prostate adenocarcinoma, the analysis achieved a sensitivity of 100% and a specificity of 90% for identifying neuroendocrine cancer.
Histone modifications
Another epigenetic alteration observed in PCa involves histone modifications. Histones, proteins in eukaryotic organisms, facilitate DNA winding into nucleosomes. These modifications, such as acetylation, methylation, phosphorylation, ubiquitination, and SUMOylation, lead to changes in chromatin structure. Such changes regulate the processes of DNA transcription, replication, and repair, or they influence the cell cycle, consequently facilitating the development of cancer.[71-73]
Growing research underscores the crucial role that histone modifications play in the onset and advancement of PCa. The levels of known histone modification markers, such as H3K27me3,[74] H4R3me2,[75] H3K9ac,[76] and H4K20me1,[77] are closely associated with the malignancy of PCa. Furthermore, these markers have been shown to effectively predict the risk of PCa recurrence, underscoring their significance in clinical prognosis.
While DNA methylation analysis is a well-established method, the analysis of histone modifications offers significant variability and adaptability, although direct examination of these modifications presents substantial challenges due to their complexity and cost. Current clinical research on histone modifications as biomarkers for PCa primarily focuses on the enzymes responsible for these modifications. For instance, the histone methyltransferase family, which is vital for gene transcription regulation, catalyzes methylation at specific gene markers in promoter regions, influencing gene expression.[78] KMT1B is upregulated in PCa cells, enhancing androgen-dependent activity through interaction with the AR.[79,80] The lysine methyltransferase SET and MYND domain-containing protein 3 are upregulated in PCa and are associated with enhanced cell migration and proliferation.[81] The increased expression of the histone-lysine N-methyltransferase SETDB1 is associated with prognosis and is thought to contribute to PCa bone metastasis through the WNT signaling pathway.[82] Protein arginine methyltransferase family members, including protein arginine N-methyltransferase 5 that catalyzes the methylation of histone arginine at H4R3, are abnormally expressed in PCa cells. This leads to the epigenetic silencing of several tumor suppressor factors, thereby facilitating the growth of PCa cells.[83] Histone acetyltransferases primarily participate in the acetylation of histones, and their increased activity can promote PCa through the acetylation of histones or transcription factors, for example, p300 and CREB-binding protein enhance the transcriptional activity of AR by acetylating it, which is a poor prognostic marker for the progression of PCa to CRPC.[84,85] At present, the clinical application of these markers is limited, mainly due to the limitations of analytical technologies. Immunohistochemistry is the only method used clinically at present, but it offers a promising avenue for further development.
RNA methylation
In addition to DNA methylation and histone modifications, in recent years, researchers have discovered numerous base modifications in mRNAs and nc-RNAs, including N6-methyladenosine (m6A), 5-methylcytosine (m5C), and N1-methyladenosine, these modifications are known as methylation of RNA.[86]
m6A is the most common form of mRNA methylation and significantly affects RNA stability and translation efficiency. In PCa, the aberrant regulation of m6A is closely associated with the onset, development, and metastasis of tumors. Research indicates that specific m6A modification patterns can serve as potential molecular markers for PCa, helping to identify different stages of the disease and predict patient prognosis.[87] Several studies have utilized RNA sequencing data from PCa tissue samples at various stages to establish m6A risk-related prognostic models for assessing the treatment outcomes and prognosis of metastatic PCa patients. These studies demonstrate that m6A scores are significantly correlated with prognostic indicators such as Gleason scores and metastatic risk, indicating the potential of m6A scoring as a molecular biomarker for evaluating prognosis through histopathological analyses.[88-90] Further, investigations reveal that the expression levels of m6A methylation regulators, including the methyltransferase-related proteins METTL3 and METTL14, are significantly positively associated with the malignancy of PCa. The strong correlation suggests that these methylation regulators may also serve as promising biomarkers for PCa, offering potential targets for diagnostic and therapeutic interventions.[91,92]
In addition, m5C is also a common RNA modification in PCa, and NSUN2, the sole m5C methyltransferase, has been proven to be an indicator of poor prognosis in PCa patients.[93] In conclusion, RNA methylation-related molecules have significant potential as diagnostic biomarkers for prognosis and disease monitoring in PCa patients. However, their detection technologies and clinical applications still require further exploration.
NC-RNA BIOMARKERS
MicroRNAs
MicroRNAs (miRNAs) are a category of small, nc-RNA molecules, typically 17–25 nucleotides long, that regulate gene expression post-transcriptionally. An increasing number of studies have investigated the potential use of miRNAs as biomarkers for cancer diagnosis, prognosis, and treatment, including PCa, and have correlated their levels with clinicopathological parameters.[94-97] In addition, miRNAs can be repeatedly extracted from various biological samples, including biopsy tissues[98] or bodily fluids[99-100] (plasma, serum, and urine), and are generally stable and resistant to various storage conditions, making them attractive molecular biomarkers for PCa.
Current research on miRNAs as biomarkers for PCa mainly focuses on two aspects: single miRNA markers and multimiRNA diagnostic models. A significant drawback of the first approach is that the results from different studies may conflict or suffer from detection errors. At present, acknowledged single miRNA markers, such as miR-21,[101] miR-34a,[102] miR-107,[103] and miR-199a-3p,[104] are significantly correlated with PCa stage and adverse outcomes, and have been validated in CTCs testing.[105,106] However, unfortunately, the diagnostic efficacy of these methods does not seem to surpass that of PSA. Several studies have demonstrated the potentially superior performance of multi-miRNA diagnostic models. For example, Porzycki et al.[107] developed and validated a composite model based on miR-21, miR-141-3p, and miR-375, achieving a sensitivity of 93% and a specificity of 63% in predicting PCa from serum samples.
A study that analyzed miRNA expression differences in urine samples from diverse groups of patients developed and validated a urinary exosome 3-miRNA diagnostic ratio model, which includes miR-24-3p, miR-222-3p, and miR-30c-5p. This model is capable of predicting biopsy results for individuals in the PSA gray area (4–10 ng/mL) and for those with a negative DRE, offering an AUC that is superior to the AUC of PSA (AUC: 0.627 vs. 0.519), effectively screening patients who need prostate biopsies when PSA tests fail.[108] Furthermore, studies have indicated that a composite model comprising miR-151-3p, miR-141, and miR-16 offers promising diagnostic efficacy in detecting mCRPC.[109] However, these multimiRNA models might necessitate further validation within larger, independent cohorts to verify their diagnostic reliability and clinical applicability.
Long nc-RNAs
Research focusing on long ncRNAs (lncRNAs) as specific diagnostic markers for PCa is currently drawing significant attention. Among them, PCA3 stands out as the most thoroughly investigated and established lncRNA biomarker for PCa to date.
In 1999, Bussemakers et al.[110] first identified the overexpression of PCA3 in PCa. Further, research revealed that PCA3 is overexpressed in 95% of PCa cases and is nearly undetectable in normal prostate tissues and other malignant non-prostate tissues, indicating that it is a highly specific biomarker for PCa.[111,112]
In 2012, the PROGENSA assay, a diagnostic test for measuring PCA3 levels in urine, was approved by the United States Food and Drug Administration for the diagnosis of PCa.[25] Among biomarkers currently used in the clinic, urinary PCA3 levels may offer greater specificity and sensitivity than PSA and similar markers.[113,114] A recent meta-analysis that assessed PCA3 found that the sensitivity and specificity of the urinary PCA3 assay were 0.65 and 0.73, respectively.[115] However, PCA3 is primarily employed to circumvent repeat biopsies in men with negative results, as there is ongoing debate regarding the ability of PCA3 expression levels to evaluate the stage and aggressiveness of PCa.[116-118]
In addition, The PCa-associated transcript-1 (PCAT1), metastasis-associated lung adenocarcinoma transcript 1 (MALAT1), and small nucleolar RNA host gene 1 have been recognized as potential biomarkers for PCa.[119] PCAT1, which is highly specific to PCa, is overexpressed in most PCa tumors, particularly in advanced, metastatic tumors. Transcriptome analyses have identified PCAT1 as the highest-ranked and most significantly upregulated lncRNA in PCa tissues.[120,121]
MALAT1 is recognized as another potential diagnostic marker for PCa. Ren et al.[122] reported that high levels of MALAT1 expression in PCa tissues were strongly associated with elevated PSA levels, higher Gleason scores, advanced tumor stages, and resistance to castration therapy. In a study involving 434 patients by Ren et al.[123], the presence of MALAT1 in urine was found to surpass the levels of PSA and free PSA before biopsy, indicating that MALAT1 is an independent marker for predicting PCa. Moreover, in patients with PSA levels exceeding 4 ng/mL, mini-RNAs derived from MALAT1 could potentially serve as a promising plasma biomarker, enhancing the diagnostic precision for predicting the outcomes of prostate biopsies.
Circular RNAs (circRNAs)
Due to their circular structure, circRNAs are highly stable against degradation by RNA exonucleases, In addition to bodily tissue, circRNAs can also be detected in human bodily fluids such as blood and urine making them ideal candidates as molecular biomarkers for PCa.[124,125]
In PCa, several circRNAs have been studied, Li et al.[126] found that circ_0044516 is overexpressed in PCa, and further, cellular experiments showed that downregulation of circ_0044516 can inhibit the proliferation and metastasis of PCa cells. In addition, the expression levels of hsa_ circ_0001275 in plasma can reflect the trends in PSA levels at specific disease time points and may be associated with resistance to enzalutamide.[127] Zhong et al.[128] identified 160 autophagy-related circRNAs and constructed a circRNA signature consisting of five circRNAs: hsa_circ_0001747, hsa_circ_0002100, hsa_circ_0000280, hsa_circ_0000437, and hsa_circ_0001085. Univariate and multivariate Cox regression analyses showed that these circRNAs are independent prognostic indicator for PCa patients. Unfortunately, although research on circRNAs as diagnostic biomarkers for PCa has made preliminary progress, there are still some challenges in their clinical application, including the standardization of circRNA detection techniques, improvements in specificity and sensitivity, and validation in large-scale population studies. A summary of these biomarkers, including oncogenic, nc-RNAs, is presented in Table 1.
Types | Biomarkers | Function | Sample types | Detection methods | References |
---|---|---|---|---|---|
Genetic Alteration | TMPRSS2-ERG | Diagnosis, Prognosis | Urine, Tissue | PCR, NGS | [21] |
PTEN | Diagnosis, Prognosis | Blood, Tissue | IHC, FISH, NGS | [34] | |
MYC | Prognosis | Blood, Tissue | FISH, NGS | [42] | |
AR | Diagnosis, Prognosis | Blood, Tissue | IHC, NGS, qRT-PCR | [49,55] | |
TP53 | Prognosis | Tissue | IHC, NGS | [148] | |
DNA Methylation | GSTP1 | Diagnosis | Blood, Tissue | MSP, qRT-PCR | [65] |
ACTL6B | Prognosis | Blood | MSP | [69] | |
TCERG1 | Prognosis | Blood, Tissue | MSP | [69] | |
Histone Modification | KMT1B | Prognosis | Tissue | Western Blot, Mass Spectrometry | [79] |
SMYD3 | Prognosis | Tissue | Western Blot, Mass Spectrometry | [81] | |
SETDB1 | Prognosis | Tissue | IHC, Western Blot | [82] | |
PRMT5 | Prognosis | Tissue | IHC, Western Blot | [83] | |
p300/CBP | Prognosis | Tissue | Chromatin Immunoprecipitation | [84] | |
METTL3 | Prognosis | Tissue | IHC, Western Blot | [91] | |
METTL14 | Prognosis | Tissue | IHC, Western Blot | [92] | |
NSUN2 | Prognosis | Tissue | IHC, Western Blot | [93] | |
miRNA | miR-21 | Diagnosis, Prognosis | Blood, Urine | qRT-PCR, Microarray | [101] |
miR-34a | Prognosis | Blood | qRT-PCR, Microarray | [102] | |
miR-107 | Diagnosis, Prognosis | Blood, Urine | qRT-PCR, Microarray | [103] | |
miR-199a-3p | Prognosis | Blood | qRT-PCR, Microarray | [104] | |
lncRNA | PCA3 | Diagnosis | Urine | qRT-PCR, Urine Tests | [25] |
SNHG1 | Diagnosis, Prognosis | Blood, Urine | qRT-PCR, NGS | [119] | |
PCAT1 | Diagnosis, Prognosis | Blood, Urine | qRT-PCR, NGS | [120] | |
MALAT1 | Diagnosis, Prognosis | Blood, Urine | qRT-PCR, NGS | [122] | |
circRNA | circ_0044516 | Prognosis | Blood | qRT-PCR, NGS | [126] |
hsa_circ_0001275 | Diagnosis, Prognosis | Blood | qRT-PCR, NGS | [127] |
PCR: Polymerase chain reaction, NGS: Next-generation sequencing, IHC: Immunohistochemistry, FISH: Fluorescence in situhybridization. MSP: Methylation-specific PCR, qRT-PCR: quantitative real time real, PCa: Prostate cancer, TMPRSS2-ERG: Transmembrane Serine Protease 2-ETS-related gene fusion, PTEN: Phosphatase and tensin homolog, MYC: Myelocytomatosis viral oncogene homolog, AR: Androgen receptor, TP53: Tumor protein p53, GSTP1: Glutathione S-transferase P1, ACTL6B: Actin-like 6B, TCERG1: Transcription elongation regulator 1, KMT1B: Lysine methyltransferase 1B, SMYD3: SET and MYND Domain Containing 3, SETDB1: SET Domain Bifurcated 1, PRMT5: Protein arginine N-methyltransferase 5, p300/CBP: E1A-associated protein p300/CREB-binding protein, METTL3: Methyltransferase like 3, METTL14: Methyltransferase like 14, NSUN2: NOP2/Sun RNA Methyltransferase 2, PCA3: Prostate cancer antigen 3, SNHG1: Small nucleolar RNA host gene 1, PCAT1: Prostate cancer massociated transcript-1, MALAT1: Metastasis-associated lung adenocarcinoma transcript 1, hsa_circ_0001275: homo sapiens circular RNA 0001275.
MOLECULAR DIAGNOSTIC TECHNIQUES
Liquid biopsy
The liquid biopsy has become a compelling and promising approach in molecular diagnostics. It serves as a minimally invasive or non-invasive technique for analyzing a range of biomarkers in blood or other body fluids for diagnosing cancer, assessing prognosis, and monitoring treatment responses. These biomarkers include CTCs, extracellular vehicles (EVs), ctDNA, and ctRNA.[129,130] Given that advanced tumor tissues are rarely obtained and may not reflect the current biological status of tumor staging, liquid biopsy is becoming an attractive resource for biomarker evaluation.
Since CTCs from primary tumors can be detected in the blood or urine of PCa patients, research on CTCs in PCa is the most advanced. CTCs have been proven to be valuable biomarkers, especially in advanced diseases such as mCRPC.[131-133] de Bono et al.[134] demonstrated that favorable CTCs counts (≤5 cells/7.5 mL of blood) could predict significantly better PFS and OS following treatment. Crucially, CTCs isolated from PCa patients can display molecular features such as increased AR copy numbers, AR mutations, PTEN loss, and TMPRSS2-ETS gene fusions, which are crucial for tumor characterization.[135] The focus of research on PCa CTCs is shifting from quantitative analysis toward the analysis of these molecular characteristics, which serve as predictive markers for diverse treatment strategies within the complex therapeutic landscape of mCRPC.
Analyzing ctDNA and ctRNA plays crucial roles in PCa research. Research indicates that ctDNA in PCa, exhibiting consistent genetic alterations such as amplification of the AR gene, speckle-type poz protein mutations, and the inactivation of genes including TP53, PTEN, APC, RB1, BRCA2, and CDKN1B, mirrors the genetic landscape of PCa. This suggests that ctDNA can aid in the molecular stratification of patients, aiding in patient prognosis and prediction.[136,137]
EVs, which are lipid bilayer-enclosed particles carrying DNA, RNA, or proteins, are naturally secreted by both healthy and cancerous cells. Exosomes, a specific subtype of EVs, are prominently featured in research related to liquid biopsy and are found in increased quantities in PCa, highlighting their potential as biomarkers for this condition.[138-140] Current research primarily centers on examining the molecular characteristics of genetic materials, such as miRNAs and lncRNAs, which are isolated from exosomes, this analysis is conducted to assess their potential as diagnostic markers.[141,142] Recently, kits designed to detect urinary exosome biomarkers, including PCA3, ERG, and sterile alpha motif pointed domain containing ETS transcription factors, have been commercialized for early PCa detection.[143]
Despite the significant promise shown by liquid biopsy in PCa management, challenges persist. Primarily, detecting low-abundance tumor-derived biomarkers in body fluids necessitates highly sensitive and specific analytical methods, such as high-throughput sequencing, which are expensive and have high technical demands, limiting their broader clinical adoption. Furthermore, establishing standardized protocols for sample collection, processing, and analysis is essential to guarantee the results’ reliability and reproducibility.[144] In addition, it is crucial to validate numerous liquid biopsy markers in larger, independent cohorts to confirm the reliability and clinical utility of their diagnostic capabilities. The application of liquid biopsy in PCa is shown in Figure 1.
![The application of the liquid biopsy in prostate cancer. (The image was created on the BioRender website and has been licensed for publication). Available from: https://app.biorender.com/ [Last accessed on 2024 Mar 28].](/content/105/2024/21/1/img/Cytojournal-21-29-g001.png)
- The application of the liquid biopsy in prostate cancer. (The image was created on the BioRender website and has been licensed for publication). Available from: https://app.biorender.com/ [Last accessed on 2024 Mar 28].
Next-generation sequencing (NGS)
Over the past decade, NGS, a technique for extensive parallel DNA sequencing, has enabled quick and thorough analyses of the genome, epigenome, and transcriptome. This is achieved through high-throughput, parallel sequencing methods. In molecular diagnostics, NGS plays a crucial role in extensively analyzing tumor genetic variations, detecting low-frequency mutations, exploring tumor heterogeneity, and identifying novel therapeutic targets.[145]
In PCa, DNA and RNA extracted from blood, biopsy, or urine samples are commonly analyzed using two main approaches: (1) whole-exome sequencing (WES) to detect mutations and copy number variations, and (2) RNA-seq to unravel gene expression patterns.[146] Algorithms that combine NGS data with clinical information have the potential to improve clinical decision-making. This integration facilitates patient stratification, diagnosis, prognosis, and the development of personalized therapy strategies. Kumar et al. were pioneers in applying NGS to mCRPC, employing WES to evaluate 16 diverse advanced metastatic tumors and 23 CaP xenografts of high-grade primary cancers from PCa patients, identified recurrent non-synonymous somatic and germline mutations in genes such as TP53, AR, PTEN, and DLK2, and detected mutations in the WNT pathway and cases with hypermutation or “ultramutation” phenotypes.[147] A retrospective study analyzing data from PCa patients who underwent NGS revealed a subset with homologous recombination repair gene alterations, making them suitable candidates for PARP inhibitor therapy.[148] This indicates that NGS testing has started to show clinical viability in PCa treatment, with the utilization of genetic alterations identified through NGS guiding treatment decisions and facilitating personalized therapy.
PROSPECTS FOR INTEGRATED MOLECULAR DIAGNOSIS AND CYTOPATHOLOGY IN PCa
For molecular diagnostics, the most important aspect is the source of the sample. Tumor cells are excellent sources of nucleic acids. With the continuous advancement of molecular technology, the ability to perform molecular diagnostics from a single cell or a small amount of circulating nucleic acids is now feasible in clinical settings. For PCa, fine-needle aspiration cytology offers a good source of samples for molecular diagnostics, including paraffin-embedded cell blocks and direct smears, which can be used for almost any type of molecular testing.[149] This means that for PCa, which is primarily diagnosed through biopsy, molecular diagnostics can go further than cytopathological diagnosis without the need for additional specimens. As cells shed from the prostate are directly released into the urethra, examining urinary cytology samples is an important method for diagnosing PCa, and it also provides an ideal source of samples for molecular diagnostics of PCa. Eskra et al.[150] collected urine from PCa patients after DRE and found that 6 out of 19 urine samples contained cancer cells, and the sensitivity reached 51% through repeated urine sampling, indicating the potential of urinary cytology for PCa diagnosis and as a source of samples for molecular diagnostics. In addition, as previously mentioned, CTCs from the blood can also provide a non-invasive cellular source for molecular diagnostics in PCa. These findings have laid a solid foundation for integrating molecular diagnostics and cytopathology in PCa.
The integration of molecular diagnostics and cytopathology in other cancers has already shown tremendous advantages. For example, in thyroid cancer, about 20% of thyroid nodules are difficult to diagnose through cytological diagnosis. Researchers use molecular diagnostic methods that combine RNA tests or DNA-RNA tests in these patients, utilizing fine-needle aspiration samples for NGS to identify genetic changes associated with thyroid cancer allowing 49% of patients with indeterminate nodules to avoid diagnostic surgery.[151] Another example is non-small cell lung cancer, where bronchial aspirates and pleural effusion cytology samples are used for disease diagnosis and to identify specific genetic and molecular abnormalities such as epidermal growth factor receptor (EGFR) mutations and anaplastic lymphoma kinase rearrangements, then targeting therapies such as EGFR and anaplastic lymphoma kinase (ALK) tyrosine kinase inhibitors are applied which are the basis of current personalized treatment for advanced lung cancer, currently, about 60% of advanced lung cancer patients benefit from this combined diagnostic-guided molecular targeted therapy.[152,153] This combined application strategy can also be applied to the diagnosis and treatment of PCa. In PCa, traditional cytopathology sometimes encounters issues with unclear boundaries and ambiguous diagnoses, at such times, changes in molecular biomarkers help to confirm diagnosis and predict disease progression. On the other hand, the discovery of molecular biomarkers allows for more personalized treatment options, such as targeted drug therapies against specific molecular targets. We believe that this integration will change the diagnostic paradigm for PCa, providing more precise and personalized methods for managing the disease.
SUMMARY
Molecular diagnostics are revolutionizing the diagnosis and treatment of PCa. As more molecular diagnostic markers are identified and diagnostic technologies advance, integrating molecular diagnostics with cytopathology into a multimodal approach will enable PCa patients to benefit from more accurate early diagnoses, precise disease classification, and effective personalized treatment strategies.
ABBREVIATIONS
PCa - prostate cancer
PTEN - phosphatase and tensin homolog
MYC - myelocytomatosis viral oncogene homolog
ADT - androgen deprivation therapy,
AR - androgen receptor
CRPC - castration-resistant prostate cancer
ETS - E-twenty six
ERG - E-twenty six-Related Gene
PSA - prostate-specific antigen
PCA3 - prostate cancer antigen 3
PI3K/AKT - the phosphoinositide 3-kinase/protein kinase B
CTCs - circulating tumor cells
Cf-DNA - circulating DNA, OS - overall survival
PFS - progression-free survival
AUC - area under the curve
GSTP1 - glutathione S-transferase P1
MSP - methylation-specific polymerase
M6A - N6-methyladenosine
M5C - 5-methylcytosine
PCAT1 - prostate cancer-associated transcript-1
MALAT1 - metastasis-associated lung adenocarcinoma transcript 1
Evs - extracellular vehicles,
NGS - next-generation sequencing
WES - whole-exome sequencing
EGFR - epidermal growth factor receptor
ALK - anaplastic lymphoma kinase.
AUTHOR CONTRIBUTIONS
JT: Drafted the manuscript and revised it critically for important intellectual content; XB: Played a key role in revising the draft; JZ and MZ: Initiated the study concept and designed the research framework. All authors contributed to editorial changes in the manuscript. All authors have participated sufficiently in the work and agreed to be accountable for all aspects of the work. All authors read and approved the final manuscript.
CONFLICT OF INTEREST
The authors declare no conflict of interest.
EDITORIAL/PEER REVIEW
To ensure the integrity and highest quality of CytoJournal publications, the review process of this manuscript was conducted under a double-blind model (authors are blinded for reviewers and vice versa) through an automatic online system.
FUNDING
This study is supported by The Key Project of Provincial Natural Science Research Project of Anhui Colleges (2022AH051147), Research Fund of Anhui Institute of translational medicine (2022zhyx-B13), and Clinical Medical Research Translational Project of Anhui Province (202204295107020031).
References
- Global cancer statistics 2020: GLOBOCAN estimates of incidence and mortality worldwide for 36 cancers in 185 countries. CA Cancer J Clin. 2021;71:209-49.
- [CrossRef] [PubMed] [Google Scholar]
- Prostate cancer review: Genetics, diagnosis, treatment options, and alternative approaches. Molecules. 2022;27:5730.
- [CrossRef] [PubMed] [Google Scholar]
- Updates in the eighth edition of the tumor-node-metastasis staging classification for urologic cancers. Eur Urol. 2018;73:560-9.
- [CrossRef] [PubMed] [Google Scholar]
- Localized and locally advanced prostate cancer: Treatment options. Oncology. 2021;99:413-21.
- [CrossRef] [PubMed] [Google Scholar]
- Management of patients with advanced prostate cancer. Part I: Intermediate-/high-risk and locally advanced disease, biochemical relapse, and side effects of hormonal treatment: Report of the advanced prostate cancer consensus conference 2022. Eur Urol. 2023;83:267-93.
- [CrossRef] [PubMed] [Google Scholar]
- Current therapy and drug resistance in metastatic castration-resistant prostate cancer. Drug Resist Update. 2023;68:100962.
- [CrossRef] [PubMed] [Google Scholar]
- Prognostic significance of morphological patterns of Gleason grade 5 prostatic adenocarcinoma diagnosed on needle biopsy. Pathology. 2021;53:199-204.
- [CrossRef] [PubMed] [Google Scholar]
- Understanding and targeting prostate cancer cell heterogeneity and plasticity. Semin Cancer Biol. 2022;82:68-93.
- [CrossRef] [Google Scholar]
- Germline DNA repair gene mutation landscape in Chinese prostate cancer patients. Eur Urol. 2019;76:280-3.
- [CrossRef] [PubMed] [Google Scholar]
- Epigenetic reprogramming during prostate cancer progression: A perspective from development. Semin Cancer Biol. 2022;83:136-51.
- [CrossRef] [PubMed] [Google Scholar]
- Role of noncoding RNA in drug resistance of prostate cancer. Cell Death Dis. 2021;12:590.
- [CrossRef] [PubMed] [Google Scholar]
- Gene of the month: TMPRSS2 (transmembrane serine protease 2) J Clin Pathol. 2020;73:773-6.
- [CrossRef] [PubMed] [Google Scholar]
- SFRP1 increases TMPRSS2-ERG expression promoting neoplastic features in prostate cancer in vitro and in vivo. Cancer Cell Int. 2020;20:312.
- [CrossRef] [PubMed] [Google Scholar]
- Transcriptome profiling of prostate cancer, considering risk groups and the TMPRSS2-ERG molecular subtype. Int J Mol Sci. 2023;24:9282.
- [CrossRef] [PubMed] [Google Scholar]
- Characterization of stage-specific tumor progression in TMPRSS2-ERG (fusion)-driven and non-fusion-driven prostate cancer in GEM models. Mol Carcinog. 2022;61:717-34.
- [CrossRef] [PubMed] [Google Scholar]
- The expression of proto-oncogene ETS-related gene (ERG) plays a central role in the oncogenic mechanism involved in the development and progression of prostate cancer. Int J Mol Sci. 2022;23:4772.
- [CrossRef] [PubMed] [Google Scholar]
- Integrative clinical transcriptome analysis reveals TMPRSS2-ERG dependency of prognostic biomarkers in prostate adenocarcinoma. Int J Cancer. 2020;146:2036-46.
- [CrossRef] [PubMed] [Google Scholar]
- Characterization of TMPRSS2-ERG fusion high-grade prostatic intraepithelial neoplasia and potential clinical implications. Clin Cancer Res. 2008;14:3380-5.
- [CrossRef] [PubMed] [Google Scholar]
- Urine TMPRSS2: ERG fusion transcript as a biomarker for prostate cancer: Literature review. Clin Genitourin Cancer. 2016;14:117-21.
- [CrossRef] [PubMed] [Google Scholar]
- Urine TMPRSS2:ERG Plus PCA3 for individualized prostate cancer risk assessment. Eur Urol. 2016;70:45-53.
- [CrossRef] [PubMed] [Google Scholar]
- Evaluation of the ETS-related gene mRNA in urine for the detection of prostate cancer. Clin Cancer Res. 2010;16:1572-6.
- [CrossRef] [PubMed] [Google Scholar]
- Prospective multicentre evaluation of PCA3 and TMPRSS2-ERG gene fusions as diagnostic and prognostic urinary biomarkers for prostate cancer. Eur Urol. 2014;65:534-42.
- [CrossRef] [PubMed] [Google Scholar]
- Re. Association between combined TMPRSS2:ERG and PCA3 RNA urinary testing and detection of aggressive prostate cancer. Eur Urol. 2018;73:301-2.
- [CrossRef] [PubMed] [Google Scholar]
- Association between combined TMPRSS2:ERG and PCA3 RNA urinary testing and detection of aggressive prostate cancer. JAMA Oncol. 2017;3:1085-93.
- [CrossRef] [PubMed] [Google Scholar]
- Mechanisms of PTEN loss in cancer: It's all about diversity. Semin Cancer Biol. 2019;59:66-79.
- [CrossRef] [PubMed] [Google Scholar]
- Deregulated PTEN/PI3K/AKT/mTOR signaling in prostate cancer: Still a potential druggable target? Biochim Biophys Acta Mol Cell Res. 2020;1867:118731.
- [CrossRef] [PubMed] [Google Scholar]
- The genomic complexity of primary human prostate cancer. Nature. 2011;470:214-20.
- [CrossRef] [PubMed] [Google Scholar]
- FABP5 inhibition against PTEN-mutant therapy resistant prostate cancer. Cancers (Basel). 2023;16:60.
- [CrossRef] [PubMed] [Google Scholar]
- A multicenter study shows PTEN deletion is strongly associated with seminal vesicle involvement and extracapsular extension in localized prostate cancer. Prostate. 2015;75:1206-15.
- [CrossRef] [PubMed] [Google Scholar]
- FISH analysis of 107 prostate cancers shows that PTEN genomic deletion is associated with poor clinical outcome. Br J Cancer. 2007;97:678-85.
- [CrossRef] [PubMed] [Google Scholar]
- PTEN loss in circulating tumour cells correlates with PTEN loss in fresh tumour tissue from castration-resistant prostate cancer patients. Br J Cancer. 2015;113:1225-33.
- [CrossRef] [PubMed] [Google Scholar]
- Discordant and heterogeneous clinically relevant genomic alterations in circulating tumor cells vs plasma DNA from men with metastatic castration resistant prostate cancer. Genes Chromosomes Cancer. 2020;59:225-39.
- [CrossRef] [PubMed] [Google Scholar]
- Clinical implications of PTEN loss in prostate cancer. Nat Rev Urol. 2018;15:222-34.
- [CrossRef] [PubMed] [Google Scholar]
- PTEN genomic deletion defines favorable prognostic biomarkers in localized prostate cancer: A systematic review and meta-analysis. Int J Clin Exp Med. 2015;8:5430-7.
- [Google Scholar]
- The MYC oncogene-the grand orchestrator of cancer growth and immune evasion. Nat Rev Clin Oncol. 2022;19:23-36.
- [CrossRef] [PubMed] [Google Scholar]
- Is Myc an important biomarker? Myc expression in immune disorders and cancer. Am J Med Sci. 2018;355:67-75.
- [CrossRef] [PubMed] [Google Scholar]
- High burden of copy number alterations and c-MYC amplification in prostate cancer from BRCA2 germline mutation carriers. Ann Oncol. 2015;26:2293-300.
- [CrossRef] [PubMed] [Google Scholar]
- Nuclear MYC protein overexpression is an early alteration in human prostate carcinogenesis. Mod Pathol. 2008;21:1156-67.
- [CrossRef] [PubMed] [Google Scholar]
- Overexpression of C-MYC oncogene in prostate cancer predicts biochemical recurrence. Prostate Cancer Prostatic Dis. 2010;13:311-5.
- [CrossRef] [PubMed] [Google Scholar]
- MEIS1 down-regulation by MYC mediates prostate cancer development through elevated HOXB13 expression and AR activity. Oncogene. 2020;39:5663-74.
- [CrossRef] [PubMed] [Google Scholar]
- NCAPG2 promotes prostate cancer malignancy and stemness via STAT3/c-MYC signaling. J Transl Med. 2024;22:12.
- [CrossRef] [PubMed] [Google Scholar]
- Copy number alterations of c-MYC and PTEN are prognostic factors for relapse after prostate cancer radiotherapy. Cancer. 2012;118:4053-62.
- [CrossRef] [PubMed] [Google Scholar]
- A novel androgen receptor antagonist JJ-450 inhibits enzalutamide-resistant mutant AR(F876L) nuclear import and function. Prostate. 2020;80:319-28.
- [CrossRef] [PubMed] [Google Scholar]
- Generation 2.5 antisense oligonucleotides targeting the androgen receptor and its splice variants suppress enzalutamide-resistant prostate cancer cell growth. Clin Cancer Res. 2015;21:1675-87.
- [CrossRef] [PubMed] [Google Scholar]
- The mutational landscape of lethal castration-resistant prostate cancer. Nature. 2012;487:239-43.
- [CrossRef] [PubMed] [Google Scholar]
- Undesirable status of prostate cancer cells after intensive inhibition of AR signaling: Post-AR era of CRPC treatment. Biomedicines. 2021;9:414.
- [CrossRef] [PubMed] [Google Scholar]
- Androgen receptor signaling in prostate cancer and therapeutic strategies. Cancers (Basel). 2021;13:5417.
- [CrossRef] [PubMed] [Google Scholar]
- Current status and future perspectives of androgen receptor inhibition therapy for prostate cancer: A comprehensive review. Biomolecules. 2021;11:492.
- [CrossRef] [PubMed] [Google Scholar]
- Circulating tumor DNA genomics correlate with resistance to abiraterone and enzalutamide in prostate cancer. Cancer Discov. 2018;8:444-57.
- [CrossRef] [PubMed] [Google Scholar]
- Rapid, ultra low coverage copy number profiling of cell-free DNA as a precision oncology screening strategy. Oncotarget. 2017;8:89848-66.
- [CrossRef] [PubMed] [Google Scholar]
- Clinical utility of androgen receptor gene aberrations in circulating cell-free DNA as a biomarker for treatment of castration-resistant prostate cancer. Sci Rep. 2019;9:4030.
- [CrossRef] [PubMed] [Google Scholar]
- Nerve density and neuronal biomarkers in cancer. Cancers (Basel). 2022;14:4817.
- [CrossRef] [PubMed] [Google Scholar]
- Prognostic and predictive value of liquid biopsy-derived androgen receptor variant 7 (AR-V7) in prostate cancer: A systematic review and meta-analysis. Front Oncol. 2022;12:868031.
- [CrossRef] [PubMed] [Google Scholar]
- Analysis of AR/ARV7 expression in isolated circulating tumor cells of patients with metastatic castration-resistant prostate cancer (SAKK 08/14 IMPROVE trial) Cancers (Basel). 2019;11:1099.
- [CrossRef] [PubMed] [Google Scholar]
- Novel epigenetic therapeutic strategies and targets in cancer. Biochim Biophys Acta Mol Basis Dis. 2022;1868:166552.
- [CrossRef] [PubMed] [Google Scholar]
- Epigenetic basis and targeting of cancer metastasis. Trends Cancer. 2022;8:226-41.
- [CrossRef] [PubMed] [Google Scholar]
- Cancer epigenetics: From mechanism to therapy. Cell. 2012;150:12-27.
- [CrossRef] [PubMed] [Google Scholar]
- Epidrugs: Targeting epigenetic marks in cancer treatment. Epigenetics. 2019;14:1164-76.
- [CrossRef] [PubMed] [Google Scholar]
- Epigenetic regulation of prostate cancer: The theories and the clinical implications. Asian J Androl. 2019;21:279-90.
- [CrossRef] [PubMed] [Google Scholar]
- Epigenetic and epitranscriptomic control in prostate cancer. Genes (Basel). 2022;13:378.
- [CrossRef] [PubMed] [Google Scholar]
- DNA methylation biomarkers distinguishing early-stage prostate cancer from benign prostatic hyperplasia. Prostate Int. 2023;11:113-21.
- [CrossRef] [PubMed] [Google Scholar]
- The epigenetic promise for prostate cancer diagnosis. Prostate. 2012;72:1248-61.
- [CrossRef] [PubMed] [Google Scholar]
- Molecular detection of prostate cancer: A role for GSTP1 hypermethylation. Eur Urol. 2004;46:660-9.
- [CrossRef] [PubMed] [Google Scholar]
- Clinical validation of an epigenetic assay to predict negative histopathological results in repeat prostate biopsies. J Urol. 2014;192:1081-7.
- [CrossRef] [PubMed] [Google Scholar]
- Glutathione-STransferase p1 gene promoter methylation in cell-free DNA as a diagnostic and prognostic tool for prostate cancer: A systematic review and meta-analysis. Int J Endocrinol. 2023;2023:7279243.
- [CrossRef] [PubMed] [Google Scholar]
- Genomic hallmarks of localized, non-indolent prostate cancer. Nature. 2017;541:359-64.
- [CrossRef] [PubMed] [Google Scholar]
- Detecting neuroendocrine prostate cancer through tissue-informed cell-free DNA methylation analysis. Clin Cancer Res. 2022;28:928-38.
- [CrossRef] [PubMed] [Google Scholar]
- Histone modifications in epigenetic regulation of cancer: Perspectives and achieved progress. Semin Cancer Biol. 2022;83:452-71.
- [CrossRef] [PubMed] [Google Scholar]
- Histone modifications and their role in epigenetics of cancer. Curr Med Chem. 2022;29:2399-411.
- [CrossRef] [PubMed] [Google Scholar]
- Epigenetic modifications of histones in cancer. Genome Biol. 2019;20:245.
- [CrossRef] [PubMed] [Google Scholar]
- Global analysis of H3K27me3 as an epigenetic marker in prostate cancer progression. BMC Cancer. 2017;17:261.
- [CrossRef] [PubMed] [Google Scholar]
- Global histone modification patterns predict risk of prostate cancer recurrence. Nature. 2005;435:1262-6.
- [CrossRef] [PubMed] [Google Scholar]
- Global levels of histone modifications predict prostate cancer recurrence. Prostate. 2010;70:61-9.
- [CrossRef] [PubMed] [Google Scholar]
- Sirtuin 7: A new marker of aggressiveness in prostate cancer. Oncotarget. 2017;8:77309-16.
- [CrossRef] [PubMed] [Google Scholar]
- The lysine demethylase, KDM4B, is a key molecule in androgen receptor signalling and turnover. Nucleic Acids Res. 2013;41:4433-46.
- [CrossRef] [PubMed] [Google Scholar]
- Androgen receptor regulation by histone methyltransferase suppressor of variegation 3-9 homolog 2 and melanoma antigen-A11. Mol Cell Endocrinol. 2017;443:42-51.
- [CrossRef] [PubMed] [Google Scholar]
- Metformin inhibits SUV39H1-mediated migration of prostate cancer cells. Oncogenesis. 2017;6:e324.
- [CrossRef] [PubMed] [Google Scholar]
- Quantitative proteomic study of human prostate cancer cells with different metastatic potentials. Int J Oncol. 2016;48:1437-46.
- [CrossRef] [PubMed] [Google Scholar]
- The PRMT5 arginine methyltransferase: Many roles in development, cancer and beyond. Cell Mol Life Sci. 2015;72:2041-59.
- [CrossRef] [PubMed] [Google Scholar]
- Acetylated histone variant H2A.Z is involved in the activation of neo-enhancers in prostate cancer. Nat Commun. 2017;8:1346.
- [CrossRef] [PubMed] [Google Scholar]
- p300 and p300/cAMP-response element-binding protein-associated factor acetylate the androgen receptor at sites governing hormone-dependent transactivation. J Biol Chem. 2000;275:20853-60.
- [CrossRef] [PubMed] [Google Scholar]
- Effects of RNA methylation on Tumor angiogenesis and cancer progression. Mol Cancer. 2023;22:198.
- [CrossRef] [PubMed] [Google Scholar]
- The functional roles of m6A modification in prostate cancer. Proteomics Clin Appl. 2023;17:e2200108.
- [CrossRef] [PubMed] [Google Scholar]
- m6A methylation regulators are predictive biomarkers for tumour metastasis in prostate cancer. Cancers (Basel). 2022;14:4035.
- [CrossRef] [PubMed] [Google Scholar]
- A prognostic signature consisting of N6-methyladenosine modified mRNAs demonstrates clinical potential in prediction of biochemical recurrence and guidance on precision therapy in prostate cancer. Transl Oncol. 2023;33:101670.
- [CrossRef] [PubMed] [Google Scholar]
- Construction and validation of N6-methyladenosine long non-coding RNAs signature of prognostic value for early biochemical recurrence of prostate cancer. J Cancer Res Clin Oncol. 2023;149:1969-83.
- [CrossRef] [PubMed] [Google Scholar]
- Silencing of METTL3 effectively hinders invasion and metastasis of prostate cancer cells. Theranostics. 2021;11:7640-57.
- [CrossRef] [PubMed] [Google Scholar]
- METTL14 promotes prostate tumorigenesis by inhibiting THBS1 via an m6A-YTHDF2-dependent mechanism. Cell Death Discov. 2022;8:143.
- [CrossRef] [PubMed] [Google Scholar]
- Multi-omics analysis of expression and prognostic value of NSUN members in prostate cancer. Front Oncol. 2022;12:965571.
- [CrossRef] [PubMed] [Google Scholar]
- MicroRNAs: Potential biomarkers for cancer diagnosis, prognosis and targets for therapy. Int J Biochem Cell Biol. 2010;42:1273-81.
- [CrossRef] [PubMed] [Google Scholar]
- Changes in circulating microRNA levels associated with prostate cancer. Br J Cancer. 2012;106:768-74.
- [CrossRef] [PubMed] [Google Scholar]
- The potential of MicroRNAs as prostate cancer biomarkers. Eur Urol. 2016;70:312-22.
- [CrossRef] [PubMed] [Google Scholar]
- miRNA-based biomarkers, therapies, and resistance in cancer. Int J Biol Sci. 2020;16:2628-47.
- [CrossRef] [PubMed] [Google Scholar]
- Circulating microRNAs as stable blood-based markers for cancer detection. Proc Natl Acad Sci U S A. 2008;105:10513-8.
- [CrossRef] [PubMed] [Google Scholar]
- Cardiospecific microRNA plasma levels correlate with troponin and cardiac function in patients with ST elevation myocardial infarction, are selectively dependent on renal elimination, and can be detected in urine samples. Cardiology. 2011;118:217-26.
- [CrossRef] [PubMed] [Google Scholar]
- High-level expression of microRNA-21 in peripheral blood mononuclear cells is a diagnostic and prognostic marker in prostate cancer. Genet Test Mol Biomarkers. 2015;19:469-75.
- [CrossRef] [PubMed] [Google Scholar]
- Effects of miR-34a on cell growth and chemoresistance in prostate cancer PC3 cells. Biochem Biophys Res Commun. 2008;377:114-9.
- [CrossRef] [PubMed] [Google Scholar]
- miR-301a expression: Diagnostic and prognostic marker for prostate cancer. Urol Oncol. 2018;36:503.e9-15.
- [CrossRef] [PubMed] [Google Scholar]
- MiR-199a-3p suppresses proliferation and invasion of prostate cancer cells by targeting Smad1. Oncotarget. 2017;8:52465-73.
- [CrossRef] [PubMed] [Google Scholar]
- Circulating RNAs in prostate cancer patients. Cancer Lett. 2022;524:57-69.
- [CrossRef] [PubMed] [Google Scholar]
- Circulating mRNAs and miRNAs as candidate markers for the diagnosis and prognosis of prostate cancer. PLoS One. 2017;12:e0184094.
- [CrossRef] [PubMed] [Google Scholar]
- Combination of three miRNA (miR-141, miR-21, and miR-375) as potential diagnostic tool for prostate cancer recognition. Int Urol Nephrol. 2018;50:1619-26.
- [CrossRef] [PubMed] [Google Scholar]
- Independent validation of a diagnostic noninvasive 3-MicroRNA ratio model (uCaP) for prostate cancer in cell-free urine. Clin Chem. 2019;65:540-8.
- [CrossRef] [PubMed] [Google Scholar]
- Plasma miRNAs as biomarkers to identify patients with castration-resistant metastatic prostate cancer. Int J Mol Sci. 2013;14:7757-70.
- [CrossRef] [PubMed] [Google Scholar]
- DD3: A new prostate-specific gene, highly overexpressed in prostate cancer. Cancer Res. 1999;59:5975-9.
- [Google Scholar]
- Diagnostic performance of PCA3 to detect prostate cancer in men with increased prostate specific antigen: A prospective study of 1,962 cases. J Urol. 2012;188:1726-31.
- [CrossRef] [PubMed] [Google Scholar]
- The role of prostate cancer antigen 3 (PCA3) in prostate cancer detection. Expert Rev Anticancer Ther. 2018;18:1013-20.
- [CrossRef] [PubMed] [Google Scholar]
- Long noncoding RNAs and prostate carcinogenesis: The missing 'linc'? Trends Mol Med. 2014;20:428-36.
- [CrossRef] [PubMed] [Google Scholar]
- Can urinary PCA3 Supplement PSA in the early detection of prostate cancer? J Clin Oncol. 2014;32:4066-72.
- [CrossRef] [PubMed] [Google Scholar]
- Comparison of diagnostic efficacy by two urine PCA3 scores in prostate cancer patients undergoing repeat biopsies. Minerva Urol Nefrol. 2019;71:373-80.
- [CrossRef] [PubMed] [Google Scholar]
- Personalized management in low-risk prostate cancer: The role of biomarkers. Prostate Cancer. 2012;2012:327104.
- [CrossRef] [PubMed] [Google Scholar]
- PCA3 in prostate cancer and tumor aggressiveness detection on 407 high-risk patients: A National Cancer Institute experience. J Exp Clin Cancer Res. 2015;34:15.
- [CrossRef] [PubMed] [Google Scholar]
- Long non-coding RNAs and prostate cancer. Cancer Sci. 2017;108:2107-14.
- [CrossRef] [PubMed] [Google Scholar]
- Updated review on analysis of long non-coding RNAs as emerging diagnostic and therapeutic targets in prostate cancers. Crit Rev Oncol Hematol. 2024;196:104275.
- [CrossRef] [PubMed] [Google Scholar]
- Clinical prospects of long noncoding RNAs as novel biomarkers and therapeutic targets in prostate cancer. Prostate Cancer Prostatic Dis. 2016;19:14-20.
- [CrossRef] [PubMed] [Google Scholar]
- Transcriptome sequencing across a prostate cancer cohort identifies PCAT-1, an unannotated lincRNA implicated in disease progression. Nat Biotechnol. 2011;29:742-9.
- [CrossRef] [PubMed] [Google Scholar]
- Long noncoding RNA MALAT-1 is a new potential therapeutic target for castration resistant prostate cancer. J Urol. 2013;190:2278-87.
- [CrossRef] [PubMed] [Google Scholar]
- Long non-coding RNA metastasis associated in lung adenocarcinoma transcript 1 derived miniRNA as a novel plasma-based biomarker for diagnosing prostate cancer. Eur J Cancer. 2013;49:2949-59.
- [CrossRef] [PubMed] [Google Scholar]
- Circular RNAs co-precipitate with extracellular vesicles: A possible mechanism for circRNA clearance. PLoS One. 2016;11:e0148407.
- [CrossRef] [PubMed] [Google Scholar]
- Circular RNAs are a large class of animal RNAs with regulatory potency. Nature. 2013;495:333-8.
- [CrossRef] [PubMed] [Google Scholar]
- Exosome circ_0044516 promotes prostate cancer cell proliferation and metastasis as a potential biomarker. J Cell Biochem. 2020;121:2118-26.
- [CrossRef] [PubMed] [Google Scholar]
- hsa_circ_0001275 is one of a number of circRNAs dysregulated in enzalutamide resistant prostate cancer and confers enzalutamide resistance in vitro. Cancers (Basel). 2021;13:6383.
- [CrossRef] [PubMed] [Google Scholar]
- Autophagy-related circRNA evaluation reveals hsa_ circ_0001747 as a potential favorable prognostic factor for biochemical recurrence in patients with prostate cancer. Cell Death Dis. 2021;12:726.
- [CrossRef] [Google Scholar]
- Liquid biopsy: From discovery to clinical application. Cancer Discov. 2021;11:858-73.
- [CrossRef] [PubMed] [Google Scholar]
- Liquid biopsy: Current technology and clinical applications. J Hematol Oncol. 2022;15:131.
- [CrossRef] [PubMed] [Google Scholar]
- Clinical phenotypes associated with circulating tumor cell enumeration in metastatic castration-resistant prostate cancer. Urol Oncol. 2015;33:110.e1-9.
- [CrossRef] [PubMed] [Google Scholar]
- Combination of circulating tumor cell enumeration and tumor marker detection in predicting prognosis and treatment effect in metastatic castration-resistant prostate cancer. Oncotarget. 2015;6:41825-36.
- [CrossRef] [PubMed] [Google Scholar]
- Circulating tumor cells in a phase 3 study of docetaxel and prednisone with or without lenalidomide in metastatic castration-resistant prostate cancer. Eur Urol. 2017;71:168-71.
- [CrossRef] [PubMed] [Google Scholar]
- Circulating tumor cells predict survival benefit from treatment in metastatic castration-resistant prostate cancer. Clin Cancer Res. 2008;14:6302-9.
- [CrossRef] [PubMed] [Google Scholar]
- Characterization of ERG, AR and PTEN gene status in circulating tumor cells from patients with castration-resistant prostate cancer. Cancer Res. 2009;69:2912-8.
- [CrossRef] [PubMed] [Google Scholar]
- Urine-and blood-based molecular profiling of human prostate cancer. Front Oncol. 2022;12:759791.
- [CrossRef] [PubMed] [Google Scholar]
- Concordance of circulating tumor DNA and matched metastatic tissue biopsy in prostate cancer. J Natl Cancer Inst. 2017;109:djx118.
- [CrossRef] [PubMed] [Google Scholar]
- Prostate extracellular vesicles in patient plasma as a liquid biopsy platform for prostate cancer using nanoscale flow cytometry. Oncotarget. 2016;7:8839-49.
- [CrossRef] [PubMed] [Google Scholar]
- Prostate-specific extracellular vesicles as a novel biomarker in human prostate cancer. Sci Rep. 2016;6:30386.
- [CrossRef] [PubMed] [Google Scholar]
- Extracellular vesicles in prostate cancer carcinogenesis, diagnosis, and management. Front Oncol. 2018;8:222.
- [CrossRef] [PubMed] [Google Scholar]
- Exosomes in diagnosis and therapy of prostate cancer. Oncotarget. 2017;8:97693-700.
- [CrossRef] [PubMed] [Google Scholar]
- Tumor-derived exosomal long noncoding RNAs as promising diagnostic biomarkers for prostate cancer. Cell Physiol Biochem. 2018;46:532-45.
- [CrossRef] [PubMed] [Google Scholar]
- Urinary biomarkers of prostate cancer. Int J Urol. 2018;25:770-9.
- [CrossRef] [PubMed] [Google Scholar]
- Liquid biopsy enters the clinic-implementation issues and future challenges. Nat Rev Clin Oncol. 2021;18:297-312.
- [CrossRef] [PubMed] [Google Scholar]
- Advances and applications in cancer diagnosis. Onco Targets Ther. 2016;9:7355-65.
- [CrossRef] [PubMed] [Google Scholar]
- Next-generation sequencing technology in prostate cancer diagnosis, prognosis, and personalized treatment. Urol Oncol. 2015;33:267.e1-13.
- [CrossRef] [PubMed] [Google Scholar]
- Exome sequencing identifies a spectrum of mutation frequencies in advanced and lethal prostate cancers. Proc Natl Acad Sci U S A. 2011;108:17087-92.
- [CrossRef] [PubMed] [Google Scholar]
- Clinical actionability and utilization of next-generation sequencing for prostate cancer in a changing treatment landscape. Front Urol. 2022;2:997396.
- [CrossRef] [Google Scholar]
- Overview of molecular testing of cytology specimens. Acta Cytol. 2020;64:136-46.
- [CrossRef] [PubMed] [Google Scholar]
- Specific detection of prostate cancer cells in urine by RNA in situ hybridization. J Urol. 2021;206:37-43.
- [CrossRef] [PubMed] [Google Scholar]
- Effectiveness of molecular testing techniques for diagnosis of indeterminate thyroid nodules: A randomized clinical trial. JAMA Oncol. 2021;7:70-7.
- [CrossRef] [PubMed] [Google Scholar]
- Acquisition and processing of endobronchial ultrasound-guided transbronchial needle aspiration specimens in the era of targeted lung cancer chemotherapy. Am J Respir Crit Care Med. 2012;185:606-11.
- [CrossRef] [PubMed] [Google Scholar]
- Biomarker-targeted therapies in non-small cell lung cancer: Current status and perspectives. Cells. 2022;11:3200.
- [CrossRef] [PubMed] [Google Scholar]